I. Introduction
II. Lamb waves in a free plate
III. Materials and methods
IV. Results and discussion
V. Conclusions
I. Introduction
Osteoporosis is characterized by low bone mass and microarchitectural deterioration of bone tissue, leading to enhanced bone fragility and a consequent increase in fracture risk.[1] Change in the cortical thickness of long bones occurring with aging and osteoporosis is also known to be a risk factor for fracture.[2] Quantitative ultrasound is now widely used for the assessment of osteoporotic fracture risk as an attractive alternative to X-ray bone densitometry because of lack of ionizing radiation, low cost, and portability. The axial transmission technique based on ultrasonic guided waves has been applied to characterize long bones (typically the tibia and the radius), which was initially proposed in the 1950s to determine fracture healing in long bones.[3] This method measures the velocity of the earliest component of the signal received along the axial direction of long bones.[4] The velocity obtained this way has been found to reflect geometric and material properties of cortical bone.[5] Recent in vitro studies have shown that the cortical shell of long bones supports the propagation of an energetic slower signal component after the first arriving signal, which can provide enhanced characterization of cortical thinning and the porosity increasing due to aging and osteoporosis.[6,7,8] Therefore, the approaches based on guided waves in long bones is expected to provide diagnostic information regarding bone quality factors such as the cortical thickness and the stiffness that cannot easily be obtained by X-ray absorptiometry techniques. However, because the overlying soft tissue of bone may affect mode generation and change the dispersive characteristics of guided waves, its effects should be considered for clinical applications in vivo.[9]
The present study aims to investigate the dependencies of phase velocities of ultrasonic guided waves on the cortical thickness in 7 soft tissue-bone mimicking phantoms consisting of acrylic plates covered by a 2 mm-thick silicone rubber layer by using the axial transmission technique with a pair of transducers with a center frequency of 200 kHz and a diameter of 12.7 mm. The Lamb wave theory for elastic wave propagation in a free plate was applied to predict the velocities of ultrasonic guided waves propagating in the 7 soft tissue-bone mimicking phantoms.
II. Lamb waves in a free plate
Lamb waves are one type of guided waves propagating in a solid plate with free boundaries in a vacuum.[10] Two types of propagating modes, symmetric and antisymmetric modes, are described by the following characteristic equations:[10]
for symmetric modes and
for antisymmetric modes, with
and
In these equations, is the plate thickness, is the angular wavenumber (where is the angular frequency and is the phase velocity of the Lamb wave), is the longitudinal velocity in the solid, and is the shear velocity in the solid. Eq. (1) gives rise to a family of waves whose motion is symmetric about the midplane of the plate (S0, S1, S2, S3, etc), while Eq. (2) gives rise to a family waves whose motion is antisymmetric about the midplane (A0, A1, A2, A3, etc). Fig. 1 shows the phase velocity dispersion curves as functions of the frequency-thickness product for the first four symmetric and antisymmetric modes for a free acrylic plate. These curves were obtained by using a longitudinal velocity of 2750 m/s and a shear velocity of 1430 m/s in acrylic.[6] As seen in Fig. 1, each Lamb mode except the two fundamental modes (S0 and A0) is found to have a cut-off frequency-thickness product below which it does not propagate. Therefore, only the S0 and the A0 modes can be excited for very thin plates or for very low frequencies. At high values of the frequency- thickness product, the phase velocities of all the Lamb modes asymptotically approach the Rayleigh velocity.
III. Materials and methods
Experimental measurements were performed on custom- made soft tissue-bone mimicking phantoms consisting of acrylic plates covered by a 2 mm-thick silicone rubber layer. Acrylic has been used as bone-mimicking phantoms in previous studies.[11,12] All acrylic plates that simulate the cortical shell of long bones had a length of 300 mm and a width of 100 mm. Seven plate thicknesses ranging from 1 mm to 4 mm were used because these dimensions are typical of those encountered in human cortical bones. Acrylic has a density of 1185 kg/m3, a longitudinal velocity of 2750 m/s, and a shear velocity of 1430 m/s. The silicone rubber layer that simulates the soft tissue surrounding the cortical shell of long bones has an attenuation coefficient of about 1 dB/cm at 1 MHz and a longitudinal velocity of 1230 m/s.[13] The attenuation coefficient of silicone rubber is very similar to that of muscle (1.09 dB/cm/MHz).[14]
Fig. 2 shows a schematic diagram of the experimental setup for ultrasound measurements by using the axial transmission technique with a pair of custom-made unfocused transducers with a center frequency of 200 kHz and a diameter of 12.7 mm. The two transducers (a transmitter and a receiver) were positioned perpendicularly to the same side of the soft tissue-bone mimicking phantom. An ultrasonic gel was applied for acoustic coupling between the transducer and the silicone rubber layer. The transmitter was excited by using a pulser/ receiver (5800PR, Panametrics, Waltham, MA). The radio- frequency (RF) signals from the receiver were digitized by using a digital oscilloscope (WS44Xs, LeCroy, Chestnut Ridge, NY) averaging over 100 waveforms and then stored on a personal computer for off-line analysis. As seen in Fig. 2, the initial distance of the transmitter and the receiver was 30 mm, which was measured from the center of the transducers. The receiver was horizontally moved along the long axis of the plate under computer control. The distance between the transmitter and the receiver increased from 30 mm to 60 mm in 0.5-mm steps. Each phantom was measured ten times.
IV. Results and discussion
Fig. 3(a) shows the time-domain signals recorded at three different transmitter-receiver distances of 35 mm, 45 mm, and 55 mm for a 2.5 mm-thick acrylic plate covered by a 2 mm-thick silicone rubber layer. The shape of the time-domain signal exhibits the presence of at least two distinct propagating waves with different velocities, the fast First Arriving Signal (FAS) and the energetic Slow Guided Waved (SGW), respectively.[2] The FAS and the SGW were consistently observed for all the soft tissue-bone mimicking phantoms. In order to determine the velocities of the FAS and SGW, the absolute amplitude of the RF signals recorded at each transmitter-receiver distance (from 30 mm to 60 mm in 0.5-mm steps) was converted into gray level with the maximum amplitude corresponding to white and plotted as a horizontal line. These horizontal lines were vertically stacked to give a diagram for visualizing propagating waves and for fitting a line to peaks within a wave packet. Fig. 3(b) shows the absolute amplitude of the RF signal as functions of time (horizontal axis) and transmitter-receiver distance (vertical axis) for a 2.5 mm-thick acrylic plate covered by a 2 mm-thick silicone rubber layer. Tracking of the FAS was performed by using the earliest detectable deviation from zero because its amplitude was generally low. The SGW was tracked by using the peak maximum within the slower wave packet. Then, we determined the phase velocities of the FAS and the SGW as the slopes of the linear regression fits to the points in each wave packet.
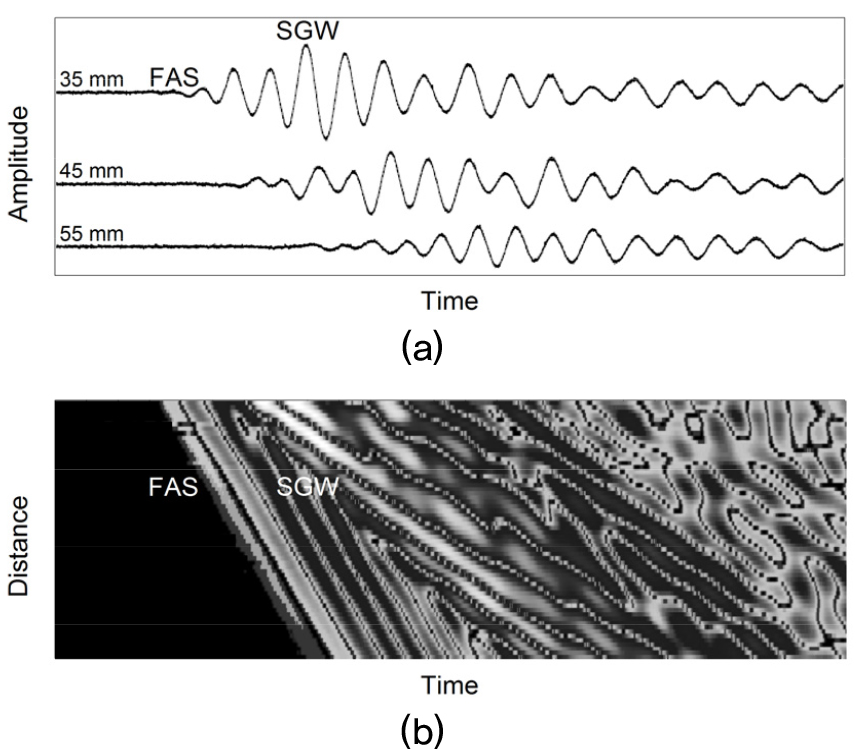
Fig. 3.
(a) Time-domain signals recorded at three different transmitter-receiver distances of 35 mm, 45 mm, and 55 mm and (b) absolute amplitude of the RF signal as functions of time (horizontal axis) and transmitter-receiver distance (vertical axis) for a 2.5 mm-thick acrylic plate covered by a 2 mm-thick silicone rubber layer.
Fig. 4 shows the experimental phase velocities of the FAS and the SGW as functions of the plate thickness for the 7 soft tissue-bone mimicking phantoms. The open circles and the asterisks represent the mean values of ten measurements for each phantom. The error bars represent the standard deviations. The theoretical phase velocities of the lowest order Lamb symmetric (S0) and the antisymmetric (A0) modes as functions of the plate thickness for a free acrylic plate were also plotted in Fig. 4. As seen in Fig. 4, the FAS velocity measured for the 7 soft tissue- bone mimicking phantoms decreased slightly with increasing thickness and exhibited good agreement with the S0 Lamb mode velocity predicted for a free acrylic plate. The SGW velocity measured for the 7 phantoms was found to increase strongly with increasing thickness, showing good agreement with the A0 Lamb mode velocity predicted for a free acrylic plate. The characteristics of Lamb waves in a plate surrounded by an external medium such as a fluid tend to be different from those of Lamb waves in a free plate because the boundary conditions may be modified by the presence of a surrounding medium. As seen in Fig. 4, the good agreement between the experimental and the theoretical velocities of the FAS and the SGW suggest that the presence of the soft tissue mimicking material (2 mm-thick silicone rubber layer) covering the acrylic plates does not influence significantly the velocity measurements. The discrepancies between the experimental and the theoretical velocities at thicknesses above 3 mm indicate that the waves contributing to the FAS change as the plate becomes thicker.[11]
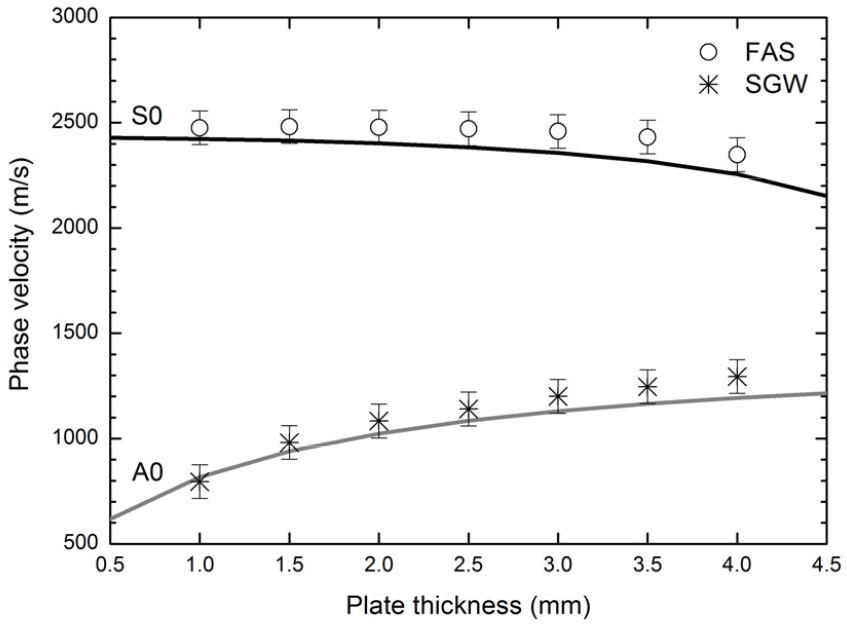
Fig. 4.
Experimental phase velocities of the FAS and the SGW as functions of the plate thickness for the 7 soft tissue-bone mimicking phantoms. The theoretical phase velocities of the lowest order Lamb symmetric (S0) and the antisymmetric (A0) modes as functions of the plate thickness for a free acrylic plate were also plotted.
In clinical examination of long bones with a commercial ultrasonometer by using the axial transmission technique, the parameter used as a discriminator of osteoporotic fracture is the FAS velocity. In the present study, the FAS velocity measured for the 7 soft tissue-bone mimicking phantoms with thicknesses less than the wavelength in acrylic (approximately 13.7 mm at 200 kHz) was found to be closely consistent with the S0 Lamb mode velocity. This is underpinned by the fact that the FAS is guided by the thickness only at wavelengths greater than the thickness.[2] For instance, Nicholson et al. reported that the FAS propagated at the longitudinal velocity in free acrylic plates with thicknesses ranging from 2 mm to 24 mm when the thickness was comparable with, or greater than, the wavelength, but its velocity decreased rapidly with decreasing thickness toward the S0 Lamb mode velocity, resulting from the interference between the lateral longitudinal wave and the Lamb modes.[11] These results suggest that the FAS velocity would be more sensitive to the thickness for long wavelengths compared to the thickness. The longitudinal velocity of 4000 m/s in cortical bone is higher than that of 2750 m/s in acrylic. This indicates that the wavelength in cortical bone (20 mm at 200 kHz) is longer than that in acrylic. Therefore, the effect of the cortical thickness on the FAS velocity in bone is expected to be more pronounced than that in the soft tissue-bone mimicking phantoms used here.
We measured a second, energetic SGW for the 7 soft tissue-bone mimicking phantoms, with velocity increasing with increasing thickness in good agreement with the A0 Lamb mode velocity predicted for a free acrylic plate. In contrast to the FAS, the SGW has shown to behave closely as expected for A0 Lamb mode, independently of the thickness-to-wavelength ratio.[2] One should note in Fig. 4 that the SGW velocity seems to be more sensitive to the thickness compared to the FAS velocity. This strongly suggests that the SGW has promising clinical potential as a parameter for characterizing cortical bone and for diagnosing osteoporosis. However, the problem arises because the SGW arriving after the FAS typically interferes with other contributions, so that a suitable signal processing technique should be implemented for consistency of mode identification and velocity measurement. Another limitation of the SGW for the clinical application is the overlying soft tissue of bone because Lamb modes are generally sensitive to the boundary conditions and the soft tissue may affect coupling of different modes and consistent mode identification. In spite of these difficulties, the use of the SGW is very attractive because its velocity exhibits the greater sensitivity to the cortical thickness compared to the FAS velocity and is strongly sensitive to the material properties throughout the entire thickness of cortical bone and thus captures pathological changes that the FAS cannot.
V. Conclusions
The present study has investigated the dependencies of phase velocities of ultrasonic guided waves on the cortical thickness in 7 soft tissue-bone mimicking phantoms consisting of acrylic plates covered by a 2 mm-thick silicone rubber layer. Two distinct propagating waves with different velocities, the FAS and the SGW, were consistently observed for all the soft tissue-bone mimicking phantoms. The FAS velocity decreased slightly with increasing thickness, whereas the SGW velocity increased strongly with increasing thickness. The FAS and the SGW velocities were found to be closely consistent with the S0 and the A0 Lamb mode velocities for a free acrylic plate, respectively, suggesting that the presence of the soft tissue mimicking material (2 mm-thick silicone rubber layer) covering the acrylic plates does not influence significantly the velocity measurements. A future study is required to address the question whether the Lamb wave model can predict with a reasonable accuracy the velocities of guided waves propagating in tube-shaped cortical bones with the overlying soft tissue.